When mitochondrial metabolism meets tumour cell radiobiology - PDF Version
Cancer is closely associated with changes in cellular bioenergetics, also called metabolic reprogramming. Under aerobic conditions, normal cells metabolise glucose to pyruvate via glycolysis in the cytoplasm. Pyruvate is then metabolised to carbon dioxide during oxidative phosphorylation in mitochondria. Under anaerobic conditions, normal cells metabolise pyruvate to lactate for rapid production of energy. In contrast, cancer cells preferentially metabolise pyruvate to lactate even under aerobic conditions, leading to increased levels of lactate and acidification of the tumour microenvironment. This glycolytic switch, also called the Warburg effect after Otto Warburg who first described this metabolic alteration in 1931, is less efficient at producing energy than oxidative phosphorylation but enables the availability of building blocks that are required for high rates of proliferation.
Aside from aerobic glycolysis, tumour cells also produce energy through upregulation of other metabolic pathways, including glutaminolysis. Aberrant activation of oncogenes and tumour-related-signalling pathways, as well as inactivation of tumour-suppressing genes, are involved in this metabolic reprogramming, which leads to specific tumour metabolic fingerprints (Oizel et al., 2017). It is now widely accepted that cancer cells follow flexible metabolic pathways that shift from glycolysis to mitochondrial oxidative phosphorylation, depending on growth states and the microenvironment. This metabolic flexibility offers an opportunity to tumour cells to survive within a microenvironment of varied oxygen levels while providing in a timely and efficient manner the cellular fuels for its biosynthetic and bioenergetic demands. These features play crucial roles in the adaptive response to metabolic stress and potentially contribute to tumour response to radiotherapy.
Tumour radiotherapy is a highly targeted and efficient way to destroy tumour cells. Its use can lead to the curing or palliation of many cancer patients. Ionising radiation (IR) kills tumour cells mainly through accumulation of DNA damage. Thus, efficiency of DNA repair is one of the most important factors that limits radiotherapy efficacy. Besides DNA lesions, IR also affects plasma membranes and subcellular organelles such as mitochondria, through the generation of reactive oxygen species (ROS) that contribute to cell death. Mitochondria are powerhouses that produce adenosine triphosphate (ATP) and carry out diverse functions for cellular energy metabolism. Mitochondrial ATP production and membrane potential require the universal cofactor nicotinamide adenine dinucleotide (NAD). As an essential coenzyme, NAD gains two electrons and one proton from substrates at multiple steps of the tricarboxylic acid (TCA) cycle and is reduced to NADH. An elegant study has used water-forming NADH oxidase as a genetic tool to induce compartment-specific modulation of NAD+/NADH ratios. It shows that the best producers of NADH are mitochondria and imbalance of the mitochondrial NADH store alters the total NADH level (Titov et al., 2016). This result may be explained by the fact that the maintenance of an optimal NAD/NADH ratio is essential for mitochondrial function, and in particular for the preservation of oxidative phosphorylation.
The importance of NAD in radiation resistance has been shown by NAD+ depletion after radiotherapy (Alano et al., 2010). Indeed, IR induces both single-strand breaks (SSB) and double-strand breaks (DSB) which activate respectively poly (adenosine diphosphate (ADP)-ribose) polymerase-1 (PARP1) and sirtuins. PARP1 breaks down NAD+ to form poly-diphosphate-ribose and nicotinamide, promoting the polyADP-ribosylation of key proteins that are required in DNA repair. Sirtuins catalyse the deacetylation of lysine residues of proteins, which results in the breakdown of NAD+ to nicotinamide and O-acetyl-ADP-ribose. In glioblastoma cell lines, high expression of NAMPT, the rate-limiting enzymes that are involved in the synthesis of NAD+, promotes both radiation resistance and survival of cancer stem-like cells (Gujar et al., 2016). Inversely, NAMPT knockdown sensitises prostate and head and neck cancer cell lines by enhancing production of deleterious ROS that are generated by exposure to IR (Lewis et al., 2019). NAD+ also contributes to the NADPH pool that is involved in numerous antioxidant pathways.
Radiation-induced oxidative stress also leads to cytoplasm-to-nucleus translocation of the transcription factor nrf2. In the nucleus, nrf2 increases the expression of genes that are involved in antioxidant responses and detoxification. A canonical gene target of nrf2 is the cytosolic flavoenzyme NAD(P)H quinone oxidoreductase (NQO1), which uses both NADH and NADPH to reduce and to detoxify quinone compounds that cause ROS generation and oxidative stress (Jaiswal et al., 2000). Nrf2 also increases the concentration of NADPH by enhancing expression of NADPH-producing enzymes such as G6PD or malic enzyme. NADPH exerts antioxidant functions by reducing oxidised glutathione and recycling peroxiredoxins.
The current NAD+-targeting strategy involves the use of β-lapachone, an NQO1 bioactivated chemotherapeutic drug, which generates strong hydrogen peroxide production, massive oxidation and multiple SSBs. This molecule exhibits tumour-selective cytotoxic effects and caspase-independent programmed necrosis in NQO1-expressing cancer cells and solid tumours. Furthermore, sub-lethal doses of β-lapachone exploit NQO1-induced massive ROS release to synergise with ROS that are directly induced by IR (Suzuki et al., 2006).
Alterations in the glycolytic metabolism modulate radiation sensitivity. A comprehensive study of the metabolic pathways in cancer patients that underwent radiotherapy has revealed an increased expression of genes that regulate mitochondrial functions and autophagy as well as a strong reliance on mitochondrial respiration and reduced dependence on aerobic glycolysis. Transcriptomic analysis has shown that expression of glutamine synthetase (GS) is significantly elevated in recurrent nasopharyngeal carcinoma samples following radiotherapy as compared to primary samples (Fu et al., 2019). When loss of function in radioresistant cancer cells increases IR-induced apoptosis, high levels of GS promote an efficient homologous recombination pathway by driving a metabolic switch toward nucleotide synthesis. Lactate production by tumour cells itself seems to regulate radiation response. In fact, high lactate content of tumours has been linked to poor outcomes, metastasis and recurrence of tumours in patients with head and neck or cervical cancer who have been treated by radiotherapy (Quennet et al., 2006).
Disruption of fundamental metabolic and signalling processes that are necessary for cancer cell survival appears to be a promising strategy to improve tumour sensitivity to radiotherapy. Among various strategies, NAD+-targeting strategies are particularly interesting since NAD metabolism is integrally connected with IR responses. While measurement of NAD still faces many technical challenges, current development of novel experimental techniques to sense and modulate NAD+/NADH, as well as methods of computational systems biology to model NAD+ metabolism, will provide great insights into these fundamental biological processes and pinpoint specific disruption of metabolic networks for improvement of radiation response.
Claire Pecqueur & François Paris
Team PETRI (Plasticity of Ecosystem from the Tumour after Radiotherapy and Immunotherapy)
Immunology and Cancer Research Centre
Nantes Angers (CRCINA) UMR Inserm
Université de Nantes
Nantes, France
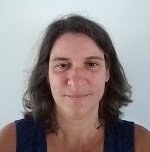
Claire Pecqueur
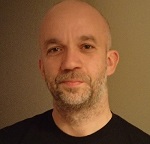
François Paris
References
• Alano CC, Garnier P, Ying W, Higashi Y, Kauppinen TM, and Swanson RA. J. Neurosci., 2010, 30(8): 2967-2978; doi.org/10.1523/jneurosci.5552-09.2010
• Gujar AD, Le S, Mao DD, Dadey DYA, Turski A, Sasaki Y, Aum D, Luo J, Dahiya S, Yuan L, Rich KM, Milbrandt J, Hallahan DE, Yano H, Tran DD, and Kim AH. PNAS, 2016, 113(51): E8247-E8256; doi.org/10.1073/pnas.1610921114
• Fu S, Li Z, Xiao L, Hu W, Zhang L, Xie B, Zhou Q, He J, Qiu Y, Wen M, Peng Y, Gao J, Tan R, Deng Y, WengL, and Sun LQ. 2019. Cell Reports. 28(5): 1136-1143. doi.org/10.1016/j.celrep.2019.07.002
• Jaiswal AK. Free Radic. Biol. & Med. 2000. 29(3–4): 254-262; doi.org/10.1016/S0891-5849(00)00306-3
• Lewis JE, Singh N, Holmila RJ, Sumer BD, Williams NS, Furdui C, Kemp ML, Boothman DA. Semin Radiat Oncol. 2019. 29(1): 6–15. doi.org/10.1016/j.semradonc.2018.10.009
• Oizel K, Chauvin C, Oliver L, Gratas C, Geraldo F, Jarry U, Scotet E, Rabe M, Alves-Guerra MC, Teusan R, Gautier F, Loussouarn D, Compan V, Martinou JC, Vallette FM, and Pecqueur C. Clin Cancer Res. 2017. 23(20):6292-6304. Doi.org/10.1158/1078-0432.
• Quennet W, Yaromina A, Zips D, Rosner A, Walenta S, Baumann M, and Mueller-Klieser W. Radiother Oncol. 2006. 81(2):130-5. doi.org/10.1016/j.radonc.2006.08.012
• Suzuki M, Amano M, Choi J, Williams BW, Ono K, and Song CW. Radiat Res. 2006. 165(5): 525–531; doi.org/10.1667/RR3554.1
• Titov DV, Cracan V, Goodman RP, Peng J, Grabarek Z, Mootha VK. Science. 2016. 352(6282): 231-235. Doi.org/10.1126/science.aad4017